Atomic Nucleus
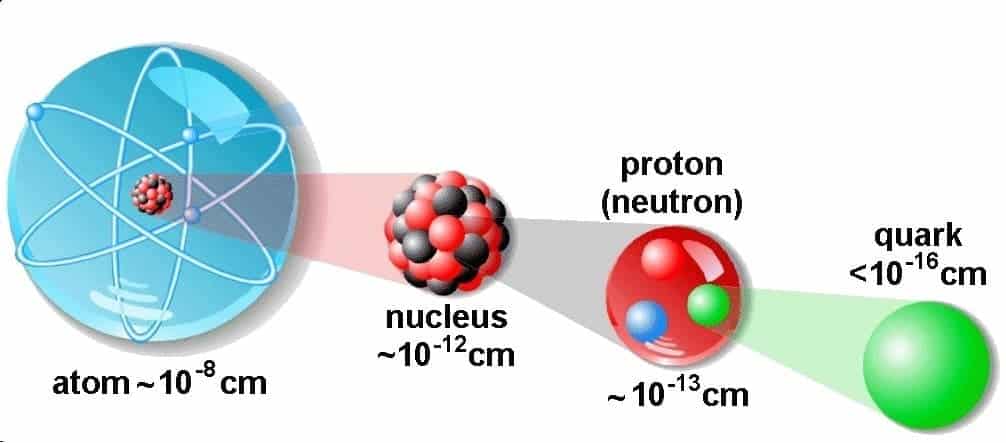
Digital lesson: Nuclear Reactions
|
---|
Nuclear Structure
Atoms consist of electrons in orbit about a central nucleus. As we have seen, the electron orbits are quantum mechanical in nature and have interesting characteristics. Little has been said about the nucleus, however. Since the nucleus is interesting in its own right, we now consider it in greater detail.
The Strong Nuclear Force and the Stability of the Nucleus
Two positive charges that are as close together as they are in a nucleus repel one another with a very strong electrostatic force. What, then, keeps the nucleus from flying apart? Clearly, some kind of attractive force must hold the nucleus together, since many kinds of naturally occurring atoms contain stable nuclei. The gravitational force of attraction between nucleons is too weak to counteract the repulsive electric force, so a different type of force must hold the nucleus together. This force is the strong nuclear force and is one of only three fundamental forces that have been discovered, fundamental in the sense that all forces in nature can be explained in terms of these three. The gravitational force is also one of these forces, as is the electroweak force.
The Mass Defect of the Nucleus and Nuclear Binding Energy
Because of the strong nuclear force, the nucleons in a stable nucleus are held tightly together. Therefore, energy is required to separate a stable nucleus into its constituent protons and neutrons. The more stable the nucleus is, the greater is the amount of energy needed to break it apart. The required energy is called the binding energy of the nucleus.
Interactive Demonstration: Binding Energy
|
Digital figure: nucleus
|
Digital figure: binding energy
|
---|
Radioactivity
When an unstable or radioactive nucleus disintegrates spontaneously, certain kinds of particles and/or high-energy photons are released. These particles and photons are collectively called "rays." Three kinds of rays are produced by naturally occurring radioactivity: $\alpha$ rays, $\beta$ rays, and $\gamma$ rays. They are named according to the first three letters of the Greek alphabet, alpha ($\alpha$), beta ($\beta$), and gamma ($\gamma$), to indicate the extent of their ability to penetrate matter. $\alpha$ rays are the least penetrating, being blocked by a thin ($\approx$ 0.01 mm) sheet of lead, whereas $\beta$ rays penetrate lead to a much greater distance ($\approx$ 0.1 mm). $\gamma$ rays are the most penetrating and can pass through an appreciable thickness ($\approx$ 100 mm) of lead.
$\alpha$ Decay
When a nucleus disintegrates and produces $\alpha$ rays, it is said to undergo $\alpha$ decay. Experimental evidence shows that a rays consist of positively charged particles, each one being the $^4 _2 He$ nucleus of helium. Thus, an $\alpha$ particle has a charge of +2e and a nucleon number of A = 4. Since the grouping of 2 protons and 2 neutrons in a $^4 _2 He$ nucleus is particularly stable, it is not surprising that an a particle can be ejected as a unit from a more massive unstable nucleus.
$\beta$ Decay
The $\beta$ rays in are deflected by the magnetic field in a direction opposite to that of the positively charged $\alpha$ rays. Consequently, these $\beta$ rays, which are the most common kind, consist of negatively charged particles or $\beta^-$ particles. Experiment shows that $\beta^-$ particles are electrons.
$\gamma$ Decay
The nucleus, like the orbital electrons, exists only in discrete energy states or levels. When a nucleus changes from an excited energy state ( denoted by an asterisk*) to a lower energy state, a photon is emitted. The process is similar to the one discussed before for the photon emission that leads to the hydrogen atom line spectrum. With nuclear energy levels, however, the photon has a much greater energy and is called a $\gamma$ ray.
The Neutrino
The nucleus, like the orbital electrons, exists only in discrete energy states or levels. When a nucleus changes from an excited energy state ( denoted by an asterisk*) to a lower energy state, a photon is emitted. The process is similar to the one discussed before for the photon emission that leads to the hydrogen atom line spectrum. With nuclear energy levels, however, the photon has a much greater energy and is called a $\gamma$ ray.
Radioactive Decay and Activity
The question of which radioactive nucleus in a group of nuclei disintegrates at a given instant is decided like the winning numbers in a state lottery: individual disintegrations occur randomly. As time passes, the number N of parent nuclei decreases. This graph of N versus time indicates that the decrease occurs in a smooth fashion, with N approaching zero after enough time has passed. To help describe the graph, it is useful to define the halflife $T_{1/2}$ of a radioactive isotope as the time required for one-half of the nuclei present to disintegrate. For example, radium $^{226} _{88}$Ra has a half-life of 1600 years, because it takes this amount of time for one-half of a given quantity of this isotope to disintegrate. In another 1600 years, one-half of the remaining radium atoms will have disintegrated. leaving only one-fourth of the original number intact. The number of nuclei present at time $t = 0$ s is $N = N_0$, and the number present at $t= T_{1/2}$ is $N = \frac{1}{2} N_0$ . The number present at $t= 2T_{1/2}$ is $N = \frac{1}{4} N_0$, and so on. The value of the half-life depends on the nature of the radioactive nucleus. Values ranging from a fraction of a second to billions of years have been found.
Radioactive Dating
One important application of radioactivity is the determination of the age of archaeological or geological samples as in the case of the mummified remains of Queen Hatshepsut. If an object contains radioactive nuclei when it is formed, then the decay of these nuclei marks the passage of time like a clock, half of the nuclei disintegrating during each half-life. If the half-life is known, a measurement of the number of nuclei present today relative to the number present initially can give the age of the sample.
Radioactive Decay Series
When an unstable parent nucleus decays, the resulting daughter nucleus is sometimes also unstable. If so, the daughter then decays and produces its own daughter, and so on, until a completely stable nucleus is produced. This sequential decay of one nucleus after another is called a radioactive decay series.
Radiation Detectors
There are a number of devices that can be used to detect the particles and photons ($\gamma$ rays) emitted when a radioactive nucleus decays. Such devices detect the ionization that these particles and photons cause as they pass through matter. The most familiar detector is the Geiger counter.
Biological Effects of Ionizing Radiation
Ionizing radiation consists of photons and/or moving particles that have sufficient energy to knock an electron out of an atom or molecule, thus forming an ion. The photons usually lie in the ultraviolet, X-ray, or $\gamma$-ray regions of the electromagnetic spectrum, whereas the moving particles can be the $\alpha$ and $\beta$ particles emitted during radioactive decay. An energy of roughly 1 to 35 eV is needed to ionize an atom or molecule, and the particles and $\gamma$ rays emitted during nuclear disintegration often have energies of several million eV. Therefore, a single $\alpha$ particle, $\beta$ particle, or $\gamma$ ray can ionize thousands of molecules.
Nuclear radiation is potentially harmful to humans because the ionization it produces can significantly alter the structure of molecules within a living cell.
Animated Physics: Alpha, Beta, and Gamma Radiation
|
Concept Map: Radioactive Decay
|
Interactive Demonstration: Nuclear Decay
|
---|---|---|
Digital figure: Radioactive Decay
|
Digital figure: radiations types
|
Virtual Investigations: Half-Life of an Element
|
Induced Nuclear Reactions
We discusses how a radioactive parent nucleus disintegrates spontaneously into a daughter nucleus. It is also possible to bring about, or induce, the disintegration of a stable nucleus by striking it with another nucleus, an atomic or subatomic particle, or a $\gamma$-ray photon. A nuclear reaction is said to occur whenever an incident nucleus, particle, or photon causes a change to occur in a target nucleus.
Nuclear Fission
In 1939 four German scientists, Otto Hahn, Lise Meitner, Fritz Strassmann, and Otto Frisch, made an important discovery that ushered in the atomic age. They found that a uranium nucleus, after absorbing a neutron, splits into two fragments, each with a smaller mass than the original nucleus. The splitting of a massive nucleus into two less massive fragments is known as nuclear fission.
Nuclear Reactors
A nuclear reactor is a type of furnace in which energy is generated by a controlled-fission chain reaction. The first nuclear reactor was built by Enrico Fermi in 1942, on the floor of a squash court under the west stands of Stagg Field at the University of Chicago. Today, there are a number of kinds and sizes of reactors, and many have the same three basic components: fuel elements, a neutron moderator, and control rods.
Nuclear Fusion
Another means of generating energy: Two nuclei with very low mass and relatively small binding energies per nucleon could be combined or "fused" into a single, more massive nucleus that has a greater binding energy per nucleon. This process is called nuclear fusion. A substantial amount of energy can be released during a fusion reaction.
Digital lab: Conservation of mass
|
||
---|---|---|
Digital figure: nuclear reactor
|
Digital figure: nuclear power plant
|
Digital simulation: A Chain Reaction
|
Elementary Particles
By 1932 the electron, the proton, and the neutron had been discovered and were thought to be nature's three elementary particles, in the sense that they were the basic building blocks from which all matter is constructed. Experimental evidence obtained since then, however, shows that several hundred additional particles exist, and scientists no longer believe that the proton and the neutron are elementary particles.
Most of these new particles have masses greater than the electron's mass, and many are more massive than protons or neutrons. Most of the new particles are unstable and decay in times between about $10^{-6}$ and $10^{-23}$ s.
Neutrinos
In 1930, Wolfgang Pauli suggested that a particle called the neutrino (now known as the "electron neutrino") should accompany the $\beta$ decay of a radioactive nucleus. As we discusses, the neutrino has no electric charge, has a very small mass (a tiny fraction of the mass of an electron), and travels at speeds approaching (but less than) the speed of light. Neutrinos were finally discovered in 1956. Today, neutrinos are created in abundance in nuclear reactors and particle accelerators and are thought to be plentiful in the universe.
Positrons and Antiparticles
The year 1932 saw the discovery of the positron (a contraction for "positive electron"). The positron has the same mass as the electron but carries an opposite charge of +e. A collision between a positron and an electron is likely to annihilate both particles, converting them into electromagnetic energy in the form of $\gamma$ rays. For this reason, positrons never coexist with ordinary matter for any appreciable length of time. The mutual annihilation of a positron and an electron lies at the heart of an important medical diagnostic technique.
The positron is an example of an antiparticle, and after its discovery scientists came to realize that for every type of particle there is a corresponding type of antiparticle. The antiparticle is a form of matter that has the same mass as the particle but carries an opposite electric charge (e.g., the electron-positron pair) or has a magnetic moment that is oriented in an opposite direction relative to the spin (e.g., the neutrino-antineutrino pair). A few electrically neutral particles, like the photon and the neutral pion ($\pi^0$), are their own antiparticles.
Muons and Pions
In 1937, the American physicists S. H. Neddermeyer (1907- 1988) and C. D. Anderson (1905- 1991) discovered a new charged particle whose mass was about 207 times greater than the mass of the electron. The particle is designated by the Greek letter $\mu$ (mu) and is known as a muon. There are two muons that have the same mass but opposite charge: the particle $\mu^-$ and its antiparticle $\mu^+$. The $\mu^-$ muon has the same charge as the electron, whereas the $\mu^+$ muon has the same charge as the positron. Both muons are unstable, and have a lifetime of $2.2 \times 10^{-6}$ s.
The Japanese physicist Hideki Yukawa (1907-1981) predicted in 1935 that pions exist, but they were not discovered until 1947. Pions come in three varieties: one that is positively charged, the negatively charged antiparticle with the same mass, and the neutral pion, mentioned earlier, which is its own antiparticle. The symbols for these pions are, respectively, $\pi^+$, $\pi^-$, and $\pi^0$. The charged pions are unstable and have a lifetime of $2.6 \times 10^{-8}$ s.
Classification of Particles
It is useful to group the known particles into three families-the bosons, the leptons, and the hadrons.
Quarks
As more and more hadrons were discovered, it became clear that they were not all elementary particles. The suggestion was made that the hadrons are made up of smaller, more elementary particles called quarks. In 1963, a quark theory was advanced independently by M. GellMann (1929- ) and G. Zweig (1937- ). The theory proposed that there are three quarks and three corresponding antiquarks, and that hadrons are constructed from combinations of these. Thus, the quarks are elevated to the status of elementary particles for the hadron family. The particles in the lepton family are considered to be elementary, and as such they are not composed of quarks.
The Standard Model
The various elementary particles that have been discovered can interact via one or more of the following four forces: the gravitational force, the strong nuclear force, the weak nuclear force, and the electromagnetic force. In particle physics, the phrase "the standard model" refers to the currently accepted explanation for the strong nuclear force, the weak nuclear force, and the electromagnetic force. In this model, the strong nuclear force between quarks is described in terms of the concept of color, and the theory is referred to as quantum chromodynarnics. According to the standard model, the weak nuclear force and the electromagnetic force are separate manifestations of a single, even more fundamental, force, referred to as the electroweak force.
Cosmology
Cosmology is the study of the structure and evolution of the universe. In this study, both the very large and the very small aspects of the universe are important. Astronomers, for example, study stars located at enormous distances from the earth, up to billions of light-years away. In contrast, particle physicists focus their efforts on the very small elementary particles ($l0^{-18}$ m or smaller) that comprise matter. The synergy between the work of astronomers and that of particle physicists has led to significant advances in our understanding of the universe. Central to that understanding is the belief that the universe is expanding, and we begin by discussing the evidence that justifies this belief.
Animated Physics: The Standard Model of Particle Physics
|
Digital figure: quarks
|
---|
You don`t have permission to comment here!
Report